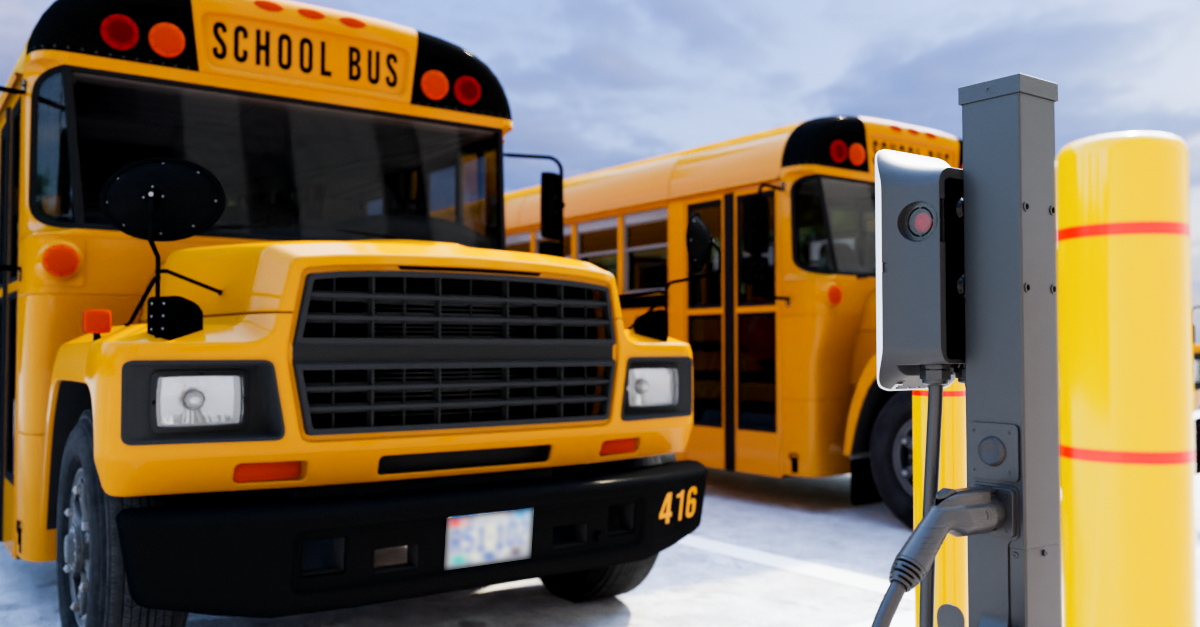
Energy Storage Technologies for Electric Vehicle Charging: A Comprehensive Technical Breakdown
As electric vehicles (EVs) become mainstream, the demand for fast, reliable, and sustainable charging infrastructure is skyrocketing. Energy storage systems (ESS) are emerging as a critical technology to support EV charging, addressing challenges like grid strain, high power demands, and renewable energy integration. By storing energy and delivering it efficiently to charging stations, ESS enhances charging performance, reduces costs, and supports a greener grid. This article dives into the technical details of energy storage technologies for EV charging, exploring their types, mechanisms, benefits, challenges, and future trends.
What is Energy Storage for EV Charging?
Energy storage systems for EV charging are technologies that store electrical energy and release it to power charging stations, particularly during peak demand or when grid supply is limited. These systems act as a buffer between the grid and chargers, enabling faster charging, stabilizing the grid, and integrating renewable energy sources like solar and wind. ESS can be deployed at charging stations, depots, or even within vehicles, offering flexibility and efficiency.
The primary goals of ESS in EV charging are:
● Grid Stability: Mitigate peak load stress and prevent blackouts.
● Fast Charging Support: Deliver high power for ultra-fast chargers without costly grid upgrades.
● Cost Efficiency: Leverage low-cost electricity (e.g., off-peak or renewable) for charging.
● Sustainability: Maximize the use of clean energy and reduce carbon emissions.
Core Energy Storage Technologies for EV Charging
Several energy storage technologies are used for EV charging, each with unique characteristics suited to specific applications. Below is a detailed look at the most prominent options:
1.Lithium-Ion Batteries
● Overview: Lithium-ion (Li-ion) batteries dominate ESS for EV charging due to their high energy density, efficiency, and scalability. They store energy in chemical form and release it as electricity via electrochemical reactions.
● Technical Details:
● Chemistry: Common types include Lithium Iron Phosphate (LFP) for safety and longevity, and Nickel Manganese Cobalt (NMC) for higher energy density.
● Energy Density: 150-250 Wh/kg, enabling compact systems for charging stations.
● Cycle Life: 2,000-5,000 cycles (LFP) or 1,000-2,000 cycles (NMC), depending on usage.
● Efficiency: 85-95% round-trip efficiency (energy retained after charge/discharge).
● Applications:
● Powering DC fast chargers (100-350 kW) during peak demand.
● Storing renewable energy (e.g., solar) for off-grid or nighttime charging.
● Supporting fleet charging for buses and delivery vehicles.
● Examples:
● Tesla’s Megapack, a large-scale Li-ion ESS, is deployed at Supercharger stations to store solar energy and reduce grid reliance.
● FreeWire’s Boost Charger integrates Li-ion batteries to deliver 200 kW charging without major grid upgrades.
2.Flow Batteries
● Overview: Flow batteries store energy in liquid electrolytes, which are pumped through electrochemical cells to generate electricity. They are known for long lifespans and scalability.
● Technical Details:
● Types: Vanadium Redox Flow Batteries (VRFB) are the most common, with zinc-bromine as an alternative.
● Energy Density: Lower than Li-ion (20-70 Wh/kg), requiring larger footprints.
● Cycle Life: 10,000-20,000 cycles, ideal for frequent charge-discharge cycles.
● Efficiency: 65-85%, slightly lower due to pumping losses.
● Applications:
● Large-scale charging hubs with high daily throughput (e.g., truck stops).
● Storing energy for grid balancing and renewable integration.
● Examples:
● Invinity Energy Systems deploys VRFBs for EV charging hubs in Europe, supporting consistent power delivery for ultra-fast chargers.
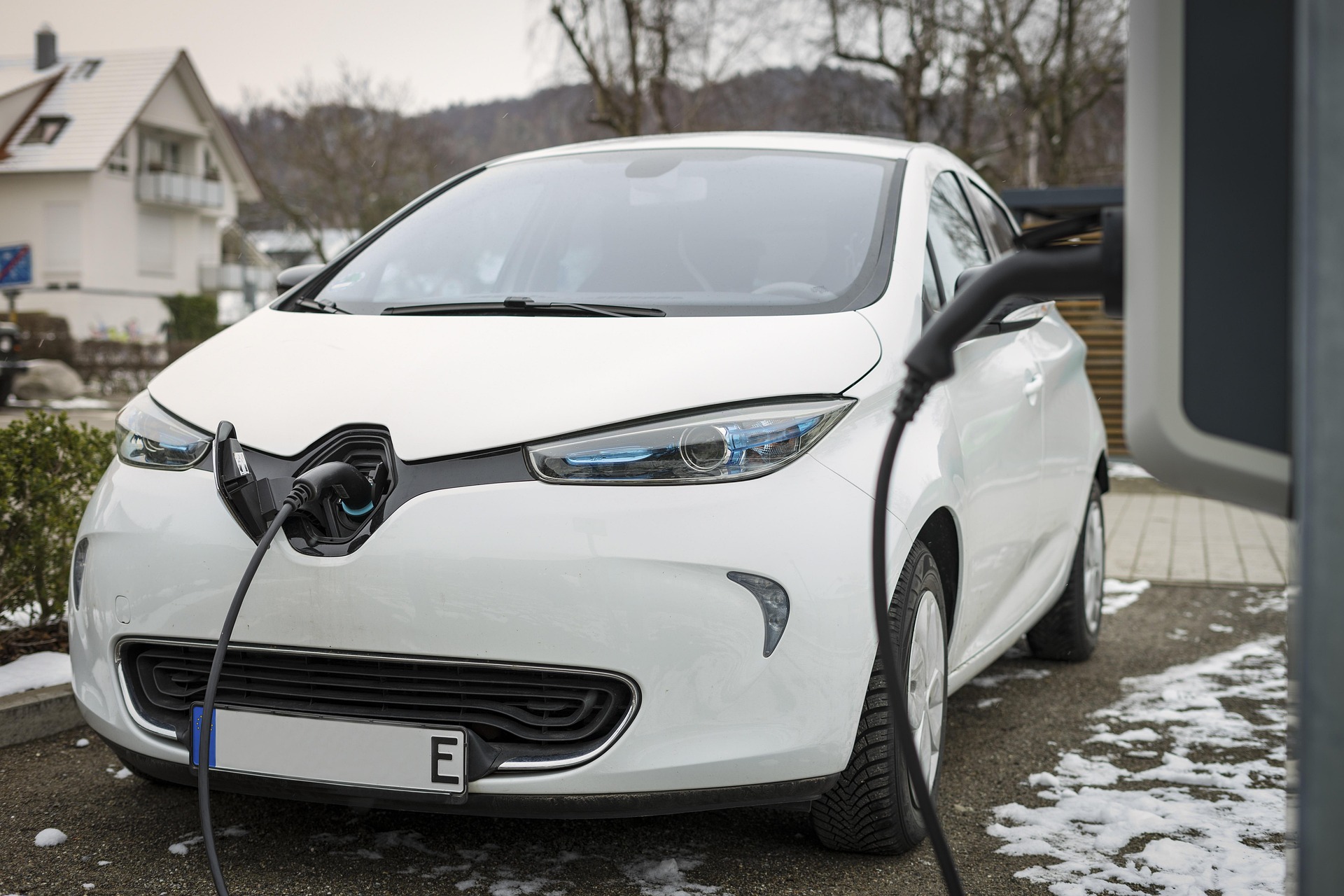
3.Supercapacitors
● Overview: Supercapacitors store energy electrostatically, offering rapid charge-discharge capabilities and exceptional durability but lower energy density.
● Technical Details:
● Energy Density: 5-20 Wh/kg, much lower than batteries.:5-20 Wh/kg.
● Power Density: 10-100 kW/kg, enabling bursts of high power for fast charging.
● Cycle Life: 100,000+ cycles, ideal for frequent, short-duration use.
● Efficiency: 95-98%, with minimal energy loss.
● Applications:
● Providing short bursts of power for ultra-fast chargers (e.g., 350 kW+).
● Smoothing power delivery in hybrid systems with batteries.
● Examples:
● Skeleton Technologies’ supercapacitors are used in hybrid ESS to support high-power EV charging in urban stations.
4.Flywheels
● Overview:
● Flywheels store energy kinetically by spinning a rotor at high speeds, converting it back to electricity via a generator.
● Technical Details:
● Energy Density: 20-100 Wh/kg, moderate compared to Li-ion.
● Power Density: High, suitable for rapid power delivery.
● Cycle Life: 100,000+ cycles, with minimal degradation.
● Efficiency: 85-95%, though energy losses occur over time due to friction.
● Applications:
● Supporting fast chargers in areas with weak grid infrastructure.
● Providing backup power during grid outages.
● Examples:
● Beacon Power’s flywheel systems are piloted in EV charging stations to stabilize power delivery.
5.Second-Life EV Batteries
● Overview:
● Retired EV batteries, with 70-80% of original capacity, are repurposed for stationary ESS, offering a cost-effective and sustainable solution.
● Technical Details:
● Chemistry: Typically NMC or LFP, depending on the original EV.
● Cycle Life: 500-1,000 additional cycles in stationary applications.
● Efficiency: 80-90%, slightly lower than new batteries.
● Applications:
● Cost-sensitive charging stations in rural or developing areas.
● Supporting renewable energy storage for off-peak charging.
● Examples:
● Nissan and Renault repurpose Leaf batteries for charging stations in Europe, reducing waste and costs.
How Energy Storage Supports EV Charging: Mechanisms
ESS integrates with EV charging infrastructure through several mechanisms:
● Peak Shaving:
● ESS stores energy during off-peak hours (when electricity is cheaper) and releases it during peak demand, reducing grid stress and demand charges.
● Example: A 1 MWh Li-ion battery can power a 350 kW charger during peak hours without drawing from the grid.
● Power Buffering:
● High-power chargers (e.g., 350 kW) require significant grid capacity. ESS provides instant power, avoiding costly grid upgrades.
● Example: Supercapacitors deliver bursts of power for 1-2 minute ultra-fast charging sessions.
● Renewable Integration:
● ESS stores energy from intermittent sources (solar, wind) for consistent charging, reducing reliance on fossil fuel-based grids.
● Example: Tesla’s solar-powered Superchargers use Megapacks to store daytime solar energy for nighttime use.
● Grid Services:
● ESS supports Vehicle-to-Grid (V2G) and demand response, allowing chargers to return stored energy to the grid during shortages.
● Example: Flow batteries in charging hubs participate in frequency regulation, earning revenue for operators.
● Mobile Charging:
● Portable ESS units (e.g., battery-powered trailers) deliver charging in remote areas or during emergencies.
● Example: FreeWire’s Mobi Charger uses Li-ion batteries for off-grid EV charging.
Benefits of Energy Storage for EV Charging
● ESS delivers high power (350 kW+) for chargers, reducing charging times to 10-20 minutes for 200-300 km of range.
● By shaving peak loads and using off-peak electricity, ESS lowers demand charges and infrastructure upgrade costs.
● Integration with renewables reduces the carbon footprint of EV charging, aligning with net-zero goals.
● ESS provides backup power during outages and stabilizes voltage for consistent charging.
● Scalability:
● Modular ESS designs (e.g., containerized Li-ion batteries) allow easy expansion as charging demand grows.
Challenges of Energy Storage for EV Charging
● High Upfront Costs:
● Li-ion systems cost $300-500/kWh, and large-scale ESS for fast chargers can exceed $1 million per site.
● Flow batteries and flywheels have higher initial costs due to complex designs.
● Space Constraints:
● Low-energy-density technologies like flow batteries require large footprints, challenging for urban charging stations.
● Lifespan and Degradation:
● Li-ion batteries degrade over time, especially under frequent high-power cycling, requiring replacement every 5-10 years.
● Second-life batteries have shorter lifespans, limiting long-term reliability.
● Regulatory Barriers:
● Grid interconnection rules and incentives for ESS vary by region, complicating deployment.
● V2G and grid services face regulatory hurdles in many markets.
● Supply Chain Risks:
● Lithium, cobalt, and vanadium shortages could drive up costs and delay ESS production.
Current State and Real-World Examples
1.Global Adoption
● Europe: Germany and the Netherlands lead in ESS-integrated charging, with projects like Fastned’s solar-powered stations using Li-ion batteries.
● North America: Tesla and Electrify America deploy Li-ion ESS at high-traffic DC fast charging sites to manage peak loads.
● China: BYD and CATL supply LFP-based ESS for urban charging hubs, supporting the country’s massive EV fleet.
2.Notable Implementations
2.Notable Implementations
● Tesla Superchargers: Tesla’s solar-plus-Megapack stations in California store 1-2 MWh of energy, powering 20+ fast chargers sustainably.
● FreeWire Boost Charger: A mobile 200 kW charger with integrated Li-ion batteries, deployed at retail sites like Walmart without grid upgrades.
● Invinity Flow Batteries: Used in UK charging hubs to store wind energy, delivering reliable power for 150 kW chargers.
● ABB Hybrid Systems: Combines Li-ion batteries and supercapacitors for 350 kW chargers in Norway, balancing energy and power needs.
Future Trends in Energy Storage for EV Charging
● Next-Generation Batteries:
● Solid-State Batteries: Expected by 2027-2030, offering 2x energy density and faster charging, reducing ESS size and cost.
● Sodium-Ion Batteries: Cheaper and more abundant than Li-ion, ideal for stationary ESS by 2030.
● Hybrid Systems:
● Combining batteries, supercapacitors, and flywheels to optimize energy and power delivery, e.g., Li-ion for storage and supercapacitors for bursts.
● AI-Driven Optimization:
● AI will predict charging demand, optimize ESS charge-discharge cycles, and integrate with dynamic grid pricing for cost savings.
● Circular Economy:
● Second-life batteries and recycling programs will reduce costs and environmental impact, with companies like Redwood Materials leading the way.
● Decentralized and Mobile ESS:
● Portable ESS units and vehicle-integrated storage (e.g., V2G-enabled EVs) will enable flexible, off-grid charging solutions.
● Policy and Incentives:
● Governments are offering subsidies for ESS deployment (e.g., EU’s Green Deal, U.S. Inflation Reduction Act), accelerating adoption.
Conclusion
Post time: Apr-25-2025